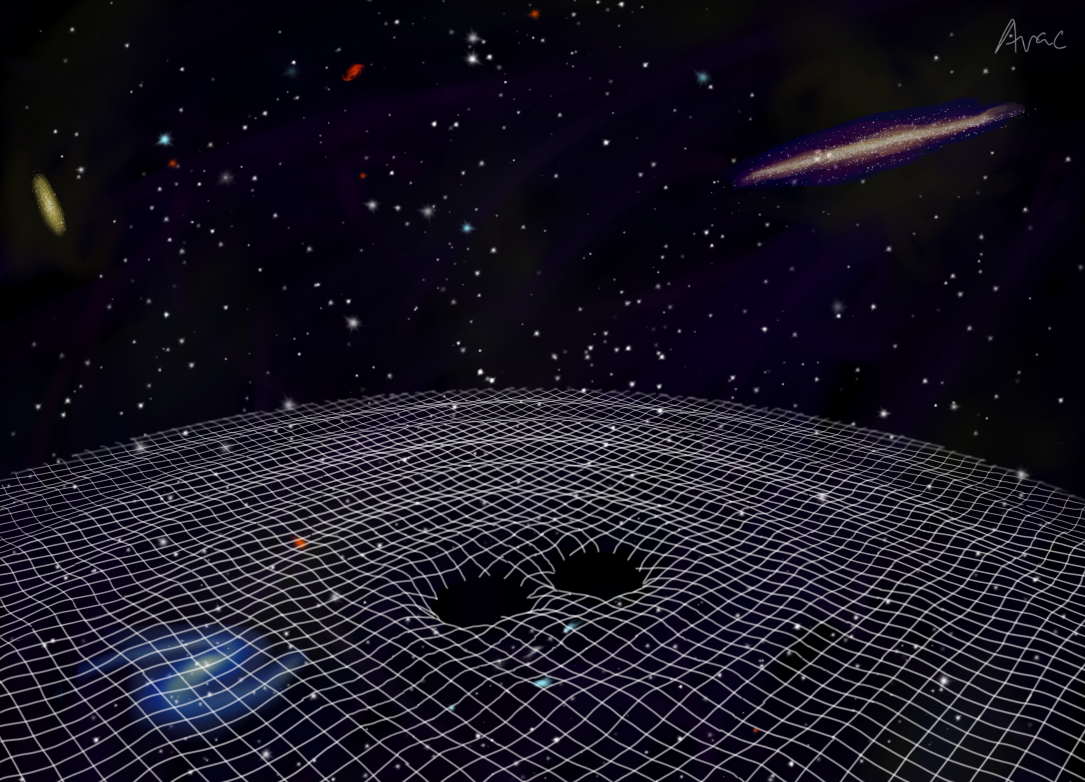
Introduction
Can you imagine what it might be like to investigate something you can’t see? Something you can’t touch and can’t even get near? How would you go about trying to learn about it?
Astrophysics is a uniquely hands-off science. It involves no microscopes, no measuring devices or samples – nothing we can hold in our hands and examine; it seems an exercise in futility. And yet, this did not deter ancient astronomers; based on the strides that they made, we have a beautiful and ever-growing understanding of the universe around us. So how is it done?
To study the universe, one has to think akin to Archimedes, exploring the law and capacity of leverage: “Give me a lever long enough, a place to stand on, and I will move the world”. In our case, it’s a matter of leveraging what, for now, we have available to us here on Earth. Our foundation to stand on is physics – using a fundamental physical understanding of how matter works and interacts. And for our lever, we primarily rely on electromagnetic radiation: light. This is our main ‘messenger’ of knowledge about the behavior of planets, stars, galaxies, and even the largest explosion in the universe: the Big Bang. If we consider what this information actually is, light amounts to extremely tiny signals emitted by stars millions of years ago, traveling a long and perilous journey through space to maybe reach us.
To understand how this ‘leveraging’ is done in modern astronomy, we can explore the science behind a recent paper published by Paynter et al. (2021), where they work to unravel a new way to study black holes, especially those that we can’t currently detect with our telescopes. We will see how the information that we receive from light is paired with our understanding of physical laws and concepts, and how a discovery can be made.
Background
Black Holes
The study of black holes and their properties has been rapidly picking up speed over the last century. From Einstein’s publication of General Relativity in 1915 on gravity influencing spacetime, to the direct detection of gravitational wave signals coming from two merging black holes in 2014, our means of understanding their traits has been growing. Over the past few decades, we have established a catalogue of indirectly detected black holes, which means that their presence and mass in a particular region has been inferred by the behavior of nearby objects such as gas, dust, or stars. We were not able to focus on the black hole itself until 2019, when the Event Horizon Telescope published results of captured light traversing the edge of the black hole and we were able to see its shape for the first time.
Black holes have quite a few interesting properties, which allow us to still study them though we can’t always directly view them. To their mass, we can ascribe a radius, though they don’t actually have a surface – ‘hole’ being the key word. We know that it’s possible for them to be spinning, and to have a charge, but beyond that, there are no other properties we can use to describe them – those three parameters are all that we have. We believe them to form during the death of a massive star, wherein the star itself is so massive that the weight of its own material causes an inward collapse with the power to create a puncture in the fabric of space. They can remain isolated as a stellar (solar)-mass black hole (2 M⊙ – 50 M⊙), or grow and evolve over time, accreting material or merging with other black holes to grow to intermediate size, hundreds and thousands times as massive as the Sun. Supermassive black holes, which can be billions of times as massive as our Sun, typically reside at the center of galaxies. Figure 2 shows a sample of smaller black holes that have been detected and measured. It can be thought of as a black hole ‘family tree’, as it shows different mergers that have taken place (two individual black holes, joined together by the white arrows) and the mass of the resulting black hole is after coalescence.
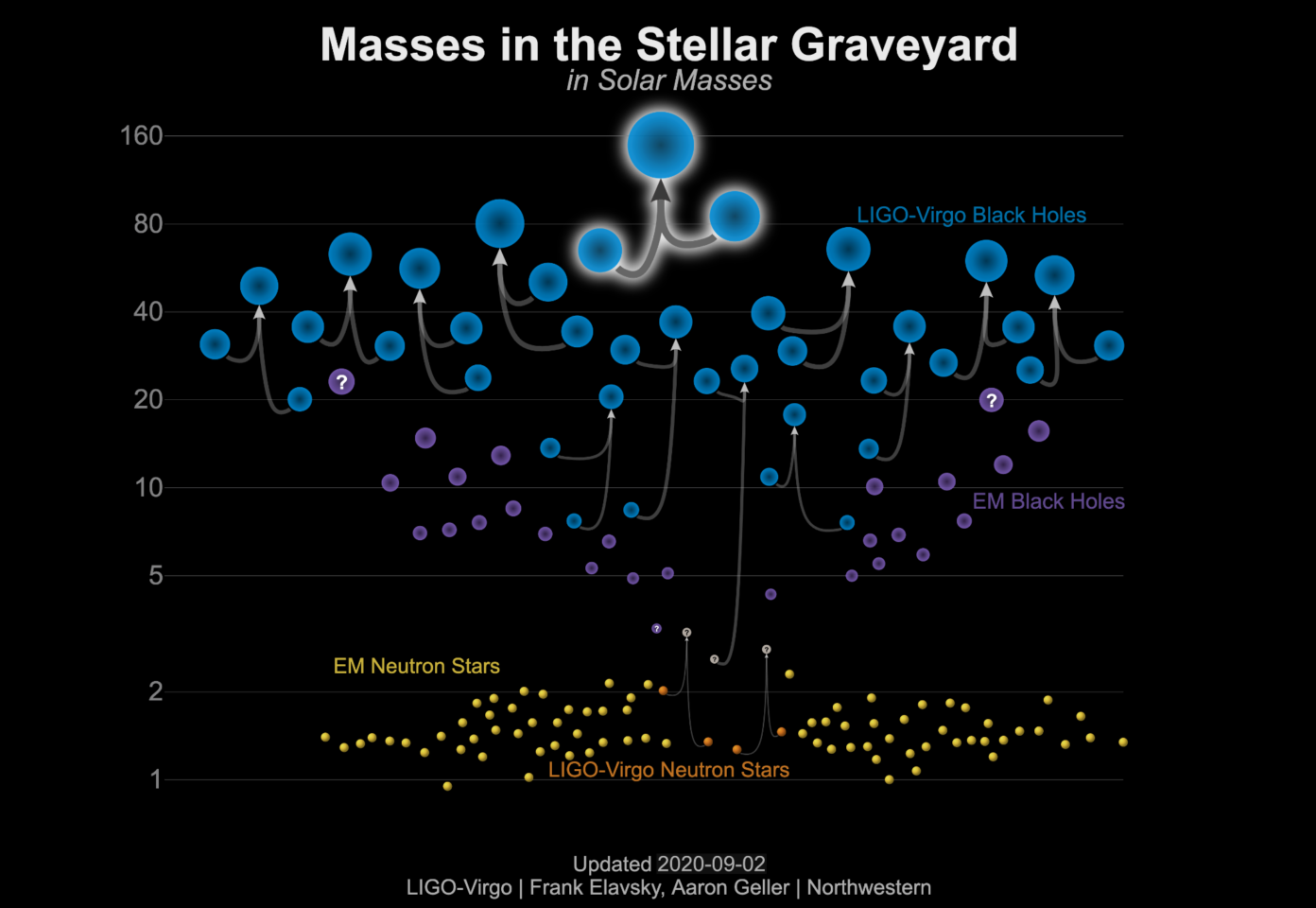
This is where we come to the first part of our particular mystery: we have not been successful in identifying the second group, the intermediate mass black holes (IMBHs). Figure 2 shows individual black holes that have been detected by different means: by gravitational wave detectors (direct detection of either black holes or neutron stars that are merging), or electromagnetically detected (black holes or neutron stars that were indirectly detected using the behavior of nearby objects, which we track with light!). For both of these classes of black holes, hundreds of sources are known and observed: stellar-mass black holes can reside in binary systems with a stellar or other compact companion (black hole, neutron star), and the supermassive black holes in galaxies we typically study are called “active galactic nuclei”. However, we don’t have an associated system or phenomenon that hosts these IMBHs. From Figure 2, we can assume that it could be common for black holes to merge and grow, and although we have a few candidates that fall at the lower end of the IMBH range, it still presents itself as a glaring gap in the black hole population.
In Figure 2, we can see that the range of detected pre-merger black hole masses goes from 1 – 80 M⊙, whereas our pre-merger IMBHs would fall within the range of 100 – 100,000 solar masses.
Shouldn’t something larger be easier to detect?
The Advanced Laser Interferometer Gravitational-wave Observatory (LIGO) and Virgo detectors are currently sensitive to mergers with a total mass ≤ 400 M⊙, which is under the amount needed for all but the very lightest IMBHs to be observed. Further, they cannot be electromagnetically detected in the same way as supermassive black holes, as IMBHs are not massive enough to instigate the same kind of behavior.
So we need to find another piece of information that can be used as leverage. The authors, Paynter et al. (2021), turned to a source of light that is both bright and constantly occurring across the universe: gamma-ray bursts.
Gamma-Ray Bursts
Gamma-ray bursts (GRBs) are unique events that also occur with the death of a star massive enough to fully collapse onto itself. They are narrow jets of energy that emerge from a dying star, flashing brightly in gamma-rays for a few seconds, and then decaying into lower energies of light. These are extremely powerful sources of emission, which release as much energy in 2-6 seconds as our Sun will in its 10 billion year lifetime.
GRBs are, by nature, isotropic, which means that we would expect them to be occurring across star forming regions of all galaxies, no matter how far away they are from us, so we can see them across the entire sky.
Unraveling the Mystery
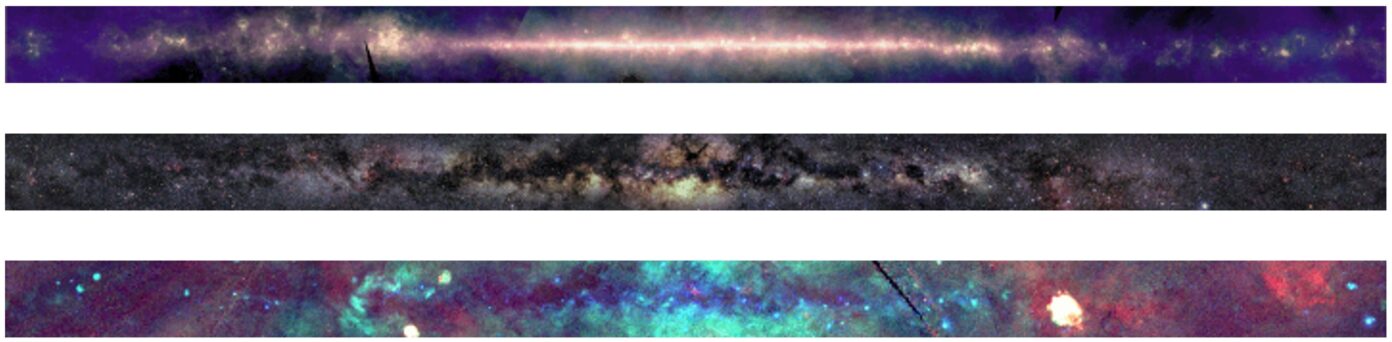
How can we then use GRBs to find our missing black holes? How are the two even related?
As discussed in the beginning of this article, electromagnetic radiation is crucial to us understanding much about a source. Further, the information we receive is far from perfect. We need to understand the different kinds of disruptions and problems that can arise with trying to obtain and interpret data from astrophysical sources. Ground based telescopes have to deal with human signal interferences, atmospheric noise, weather, maintenance – and space-based telescopes are beyond our reach if something malfunctions. On top of all of these difficulties within our immediate environment, there’s also space to consider. The solar system is along one of the spiral arms of the Milky Way, which is a region of our galaxy that is full of diffuse gas and dust. While that’s very good for forming stars, it also blocks a lot of light from reaching us by absorbing it. In general, incoming light might be absorbed, scattered and redirected on its way to us and this can restrict our view of the universe, depending on how and where we are looking. Light of different wavelengths will, for example, interact differently with clouds of gas in the Milky Way, which is best seen in Figure 3.
This shows the central band of the galaxy in three different wavelengths: infrared, optical, and X-ray light. In the case of the infrared, we get to see the defined structure of the galactic plane, with a high concentration of stars. In the optical, the clouds of gas are defined as they have absorbed the lower-energy optical light as it was traveling towards us. And in the X-rays, we’re able to identify many individual sources across the galaxy that were hidden from us before. Piecing together the behavior of stars and galaxies involves utilizing different wavelengths and having a strong understanding of how light can be disrupted on its path to us.
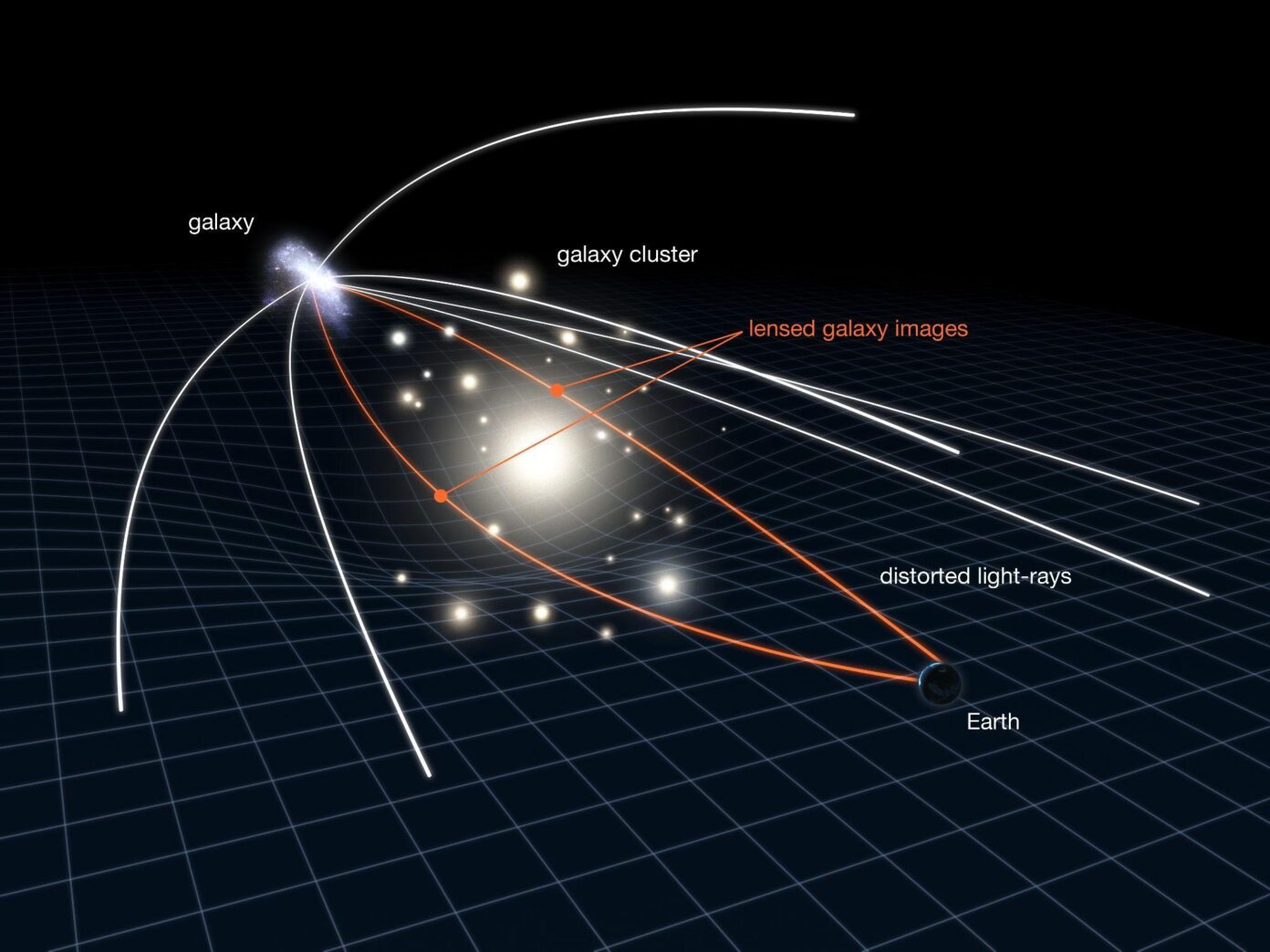
In one instance, however, the distortion of light traveling towards us is more of an aid than a hindrance. This is the case of gravitational lensing: massive objects distorting the fabric of space around them (Einstein’s paper from 1915!). When light is emitted from a source, it will propagate through space in straight lines, following the most direct path. However, if it comes across a massive enough object bending spacetime, the light will be forced along a curved path – see Figure 4 for a diagram of this mechanism.
We can see this effect happening with galaxies and black holes where long streaks will appear in galaxy field images (Figure 5). In some instances, the alignment of the massive object, incoming light, and Earth is exact, and the light will be bent into a circle, which is known as an Einstein ring. This is shown in Figure 6, where the central red galaxy, LRG 3-757, has distorted the light from a further galaxy into a nearly perfect ring.
From the tenets of general relativity, we are able to infer properties of the massive object based on how much it is distorting spacetime, giving us the lensed effect. From the angular size of the ring, we can derive the mass of the object if we are able to determine the distance between the object and the Earth, and between the object and the source.
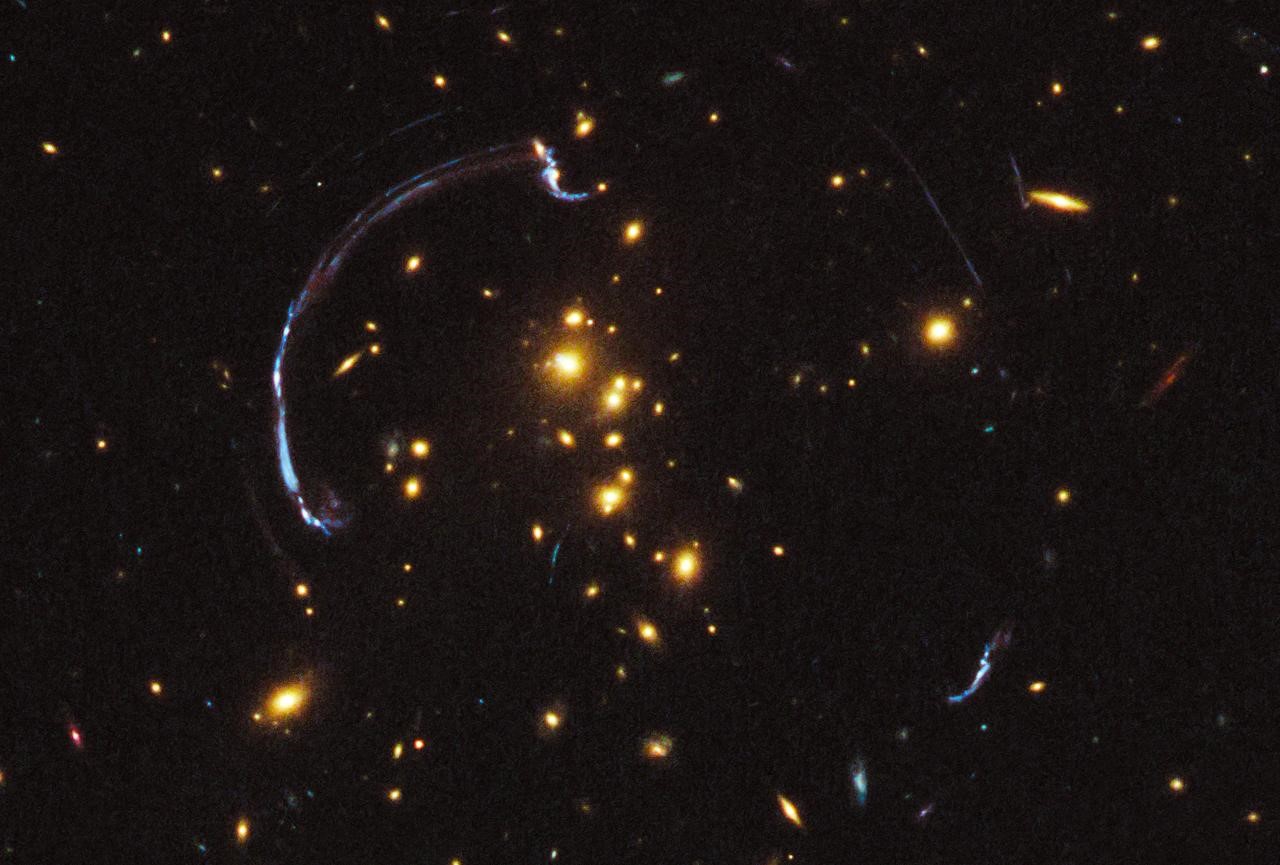
Recalling back to the different ways in which we observe the universe, there is more than one way for us to determine whether a signal has been gravitationally lensed, aside from observing it in optical images. For a field of photons traveling towards us, if a portion of them encounter a massive object (our black holes), traveling along the curve created in spacetime will be slower than following a straight path, and the arrival time of the ‘curved’ light is noticeably later than that of the rest.
If we consider our gamma-ray burst signals in particular with this lensing effect, we can think of it similar to someone standing behind a pillar with a flashlight. In this scenario, the pillar is our black hole, and the flashlight is our gamma-ray burst. If you’re standing on the other side, and you see someone switch flashlight on and off, you’ll glimpse the edges of the light coming around the pillar almost immediately. However, in the case of a black hole, we know that space will not be acting exactly similarly, that it becomes curved and warped as you approach it. So, the closer that light gets to the black hole, or the more that it has to traverse this warped spacetime, the longer it will take to reach us. Back to the example with the flashlight, this would be akin to the person on the other side switching it on, and then you are only able to see the broad edges of the flashlight’s beam away from the pillar before it illuminates anything nearer to its surface.
If we had a measuring device for photons with us on our side of the pillar, we might see something like an initial spike in the arriving photons that traveled wider around the pillar, and then a few seconds later, another spike for the photons that had to travel close to the pillar. This is exactly what we can do with gamma-ray bursts.
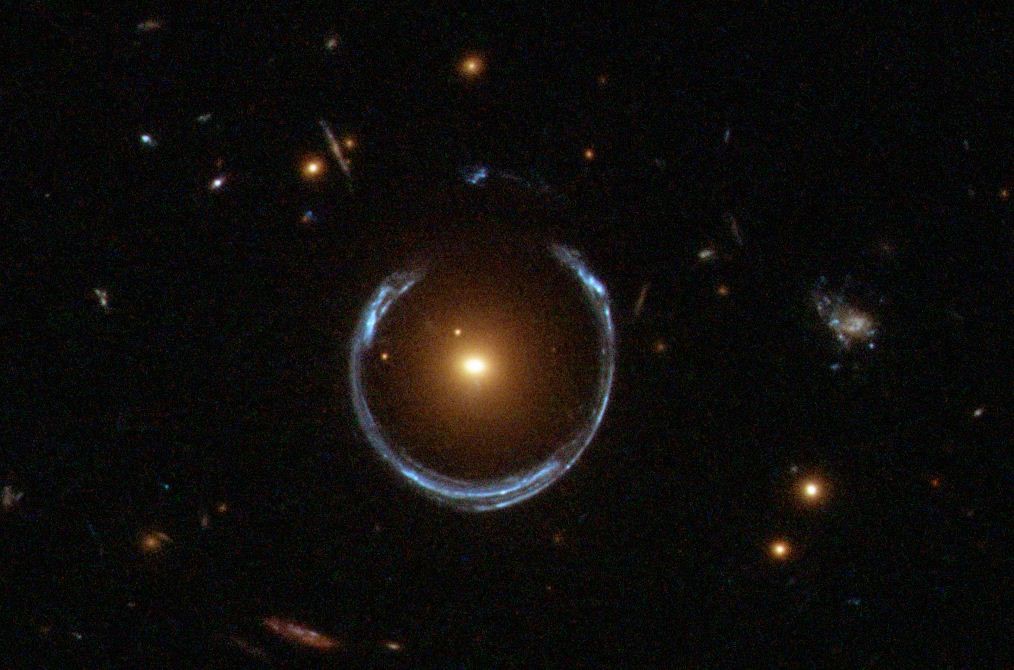
Concerning the Paper
We have now pieced together a means of extracting knowledge out of the information coming to us. We have identified frequent, isotropic sources in the sky (our GRBs) and know that if they’re traveling towards us from all distances, they can encounter massive objects (in particular, black holes) along the way.
The study that we have been focusing on by Paynter et al. (2021) searched through a catalogue of 2,700 GRBs to search for any instances of gravitational lensing via the delay in arrival times. This would appear as the second, smaller ‘flare’ in the data after the initial emission received, for the portion of the light that traveled slower around the curve of the massive object. They were able to find a very clear case of possible gravitational lensing in the signal from GRB 950830, a burst detected in 1995 by the Burst and Transient Spectrometer Experiment.
Several different energies are shown in Figure 7, and the two distinct flares are easily seen at 0, 0.4 s. This difference in timing allows for them to determine an intervening mass of ≈ 5.5 × 104 solar masses. This falls into the range of what they were looking for: an IMBH!
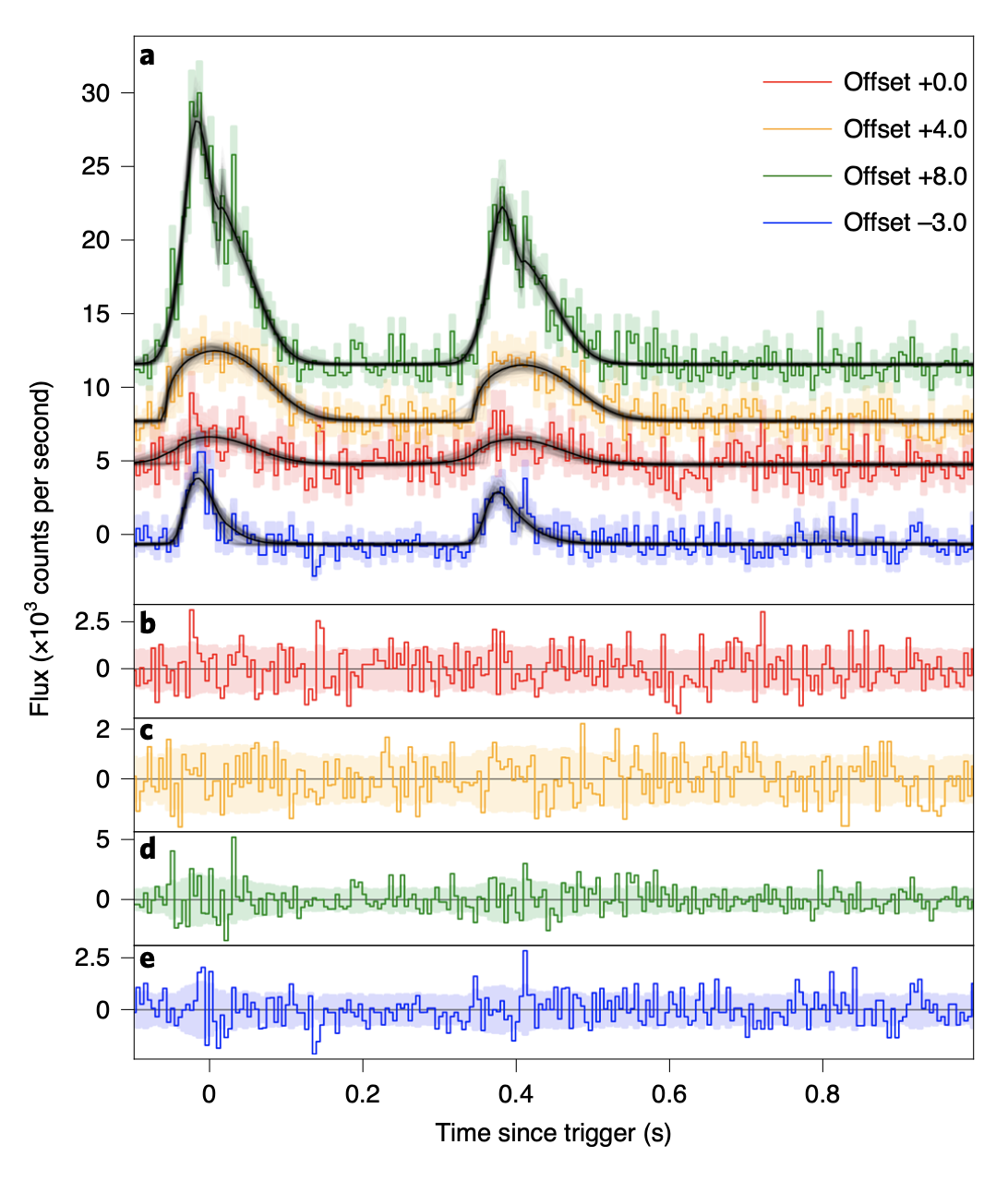
Conclusion
We have to acknowledge the serendipity of obtaining this result: 10 billion years ago in a distant binary system, two neutron stars merged into one, emitting a GRB. The light from that burst traveled toward us, and along the way, may have come across a black hole that just so happens to be in a population we cannot currently detect.
So how can we detect more?
Although it is impossible for us to say with complete certainty, the repeated signal detected in GRB 950803 has been strongly favored to be caused by a lensing event. This relied on the fact that there was a substantial amount of data collected on the burst itself, which meant that it was possible to apply our current understanding of normal (un-lensed) GRB signals and verify that it was a GRB, and not some unknown phenomenon. Further, it was possible to determine an accurate distance for the source of the gamma-rays as well as the distance to the massive object acting as the lens. This allowed the authors to verify that the two signals were from the same distance, at the same location in the sky, and therefore more unlikely to be two different GRBs or sources of gamma-rays.
This GRB was detected in 1995, and was identified after a careful catalogue search. Therefore, it’s likely that we have detected other GRBs that have been gravitationally lensed as well and show a delay in their signals, but it is important to be more cautious with drawing conclusions if the data is less clean or plentiful. With approximately one GRB detected per day, we should be able to keep investigating gravitational lensing instances in our catalogues.
Ultimately, however, the possible identification of an IMBH, even through a single source, is extremely valuable. It helps us in starting to pin down the seeds of such a population, and understand the large-scale picture of black hole growth and evolution. For now what we can do, by considering the serendipity of detecting an IMBH, is make estimates on how common black holes and specifically IMBHs would need to be throughout galaxies across the universe, based on all of our black hole observations and understanding of stellar evolution. The authors make an estimate on the present day number density of IMBHs in the 104 – 105 M⊙ range: around~ 2.3 × 103 per cubic megaparsec, which would mean that there should be about~ 4.6 × 103 IMBHs residing in the neighborhood of our Milky Way galaxy.
So, while the next generation of gravitational wave detectors is built, we can continue to investigate the universe as we always have: leveraging our position here on Earth.