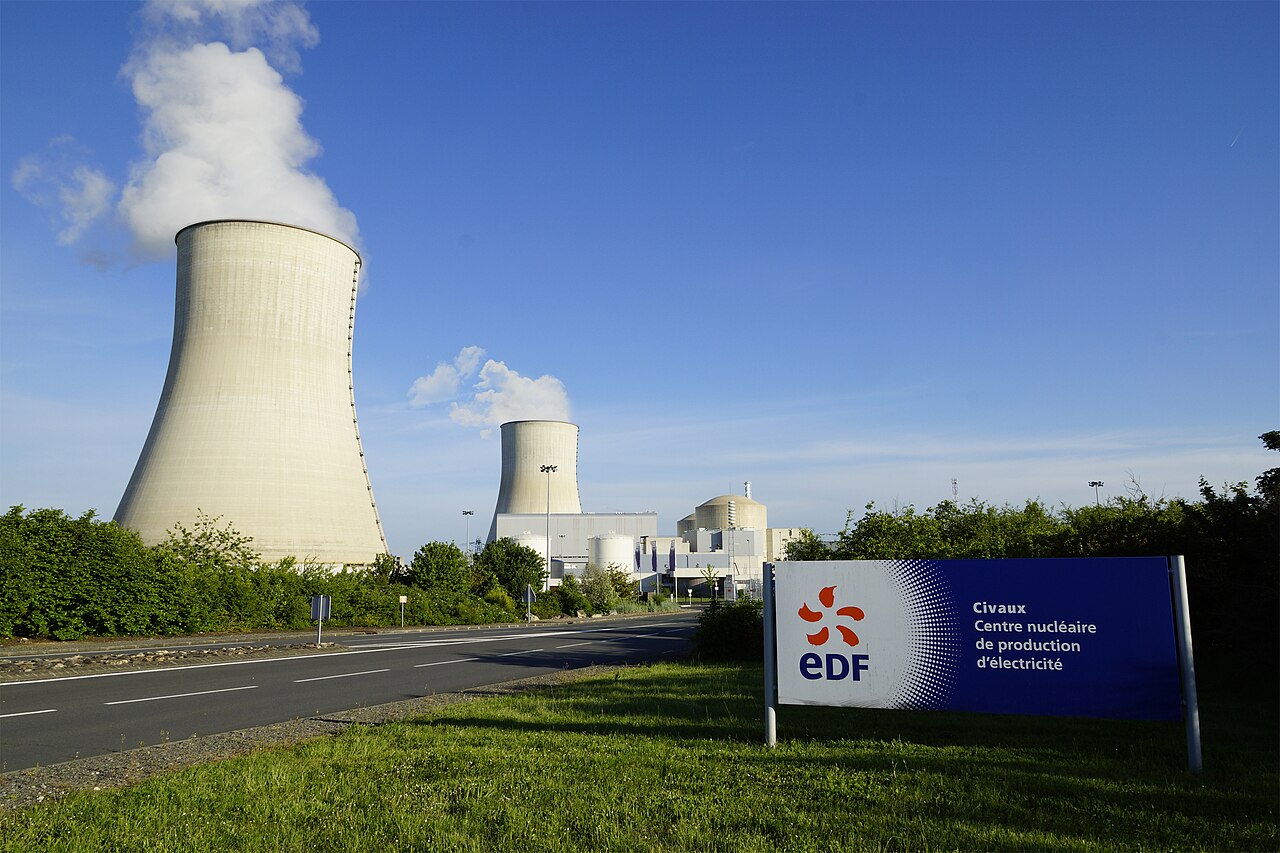
How ’ghost’ particles allow us to monitor nuclear reactors
Since you are reading this article, I bet you have also seen the movie Oppenheimer, which was released in 2023. This movie describes how, in a project led by Rober Oppenheimer, nuclear technology was developed to create a weapon that would end World War II. The nuclear bombs that were detonated over Hiroshima and Nagasaki in 1945 demonstrated the immense devastating power of nuclear weapons.
However, the story of nuclear technology did not stop here. After the war, researchers shifted their focus to peaceful applications, exploring reactors for commercial energy production. In 1951, for the first time, electricity was generated by a nuclear reactor, EBR-1. Up to this day, nuclear reactors are among the main energy sources in the world, generating and offering a low carbon alternative to fossil fuels. However, alongside these benefits, the underlying threat remains: the dual-use nature of nuclear technology means that the same processes that fuel power plants, can also create material for nuclear weapons.
To prevent this from happening, the world relies on strict monitoring of nuclear power plants and strict international agreements. The International Atomic Energy Agency (IAEA), established in 1957, is an international organization that ensures that nuclear materials and technologies are not used for military purposes. Their activities include, among others, regular inspections, inventory checks, and material sampling and analysis.
All of these methods require direct access to the reactor. What if there were a way to monitor nuclear power plants without setting foot inside the reactor? What if we could ensure the safe and peaceful use of nuclear reactors from hundreds of kilometers away from the power plant?
Reactor processes
Nuclear energy is energy that is released from nuclei. When heavy nuclei inside the nuclear fuel absorb a neutron, they can undergo nuclear fission. This is a process in which the atomic nucleus is split into smaller fragments, called fission products, thereby releasing energy. In the nuclear fission process, neutrons are released, which can in turn be absorbed by other heavy nuclei, again resulting in nuclear fission. This results in a chain reaction releasing a large amount of energy.
Let us now look at the differences between nuclear energy generation and nuclear weapon production. A nuclear weapon is designed for an uncontrolled, rapid chain reaction, releasing an immense amount of energy in a fraction of a second, whereas nuclear energy production requires a steady, controlled release of heat from the fission over a long period of time. Usually, nuclear fission starts with uranium, which is found in nature as a mixture of two isotopes: uranium-235 (U-235) and uranium-238 (U-238). U-235, the isotope with the smallest number of neutrons, is the relevant isotope for nuclear technology, as it easily undergoes fission. However, U-235 makes up less than 1% of the world’s natural uranium. For this reason, in order to produce nuclear energy or nuclear weapons, one needs to increase the proportion of U-235 through a process called enrichment. Nuclear weapon production requires a mixture with more than 90% U-235, whereas nuclear energy generation only requires 3-5% of U-235. This is because the high percentage of U-235 will result in a much more rapid and uncontrolled chain reaction than a low percentage of U-235.
Now that the differences between nuclear energy generation and nuclear weapon production have been enlightened, we will take a look at how these differences can help monitor reactors from a distance. In order to do this, we first have to talk about neutrinos and how they are relevant in monitoring nuclear reactors.
Reactor neutrino detection
Neutrinos are fascinating particles, sometimes referred to as ghost particles, as they are so small and elusive that, every second, trillions of them pass through your body without a trace. This is because neutrinos are very weakly interacting particles, as a result of which they can travel large distances without any interaction. Due to this property, neutrinos are very hard, but certainly not impossible, to detect.
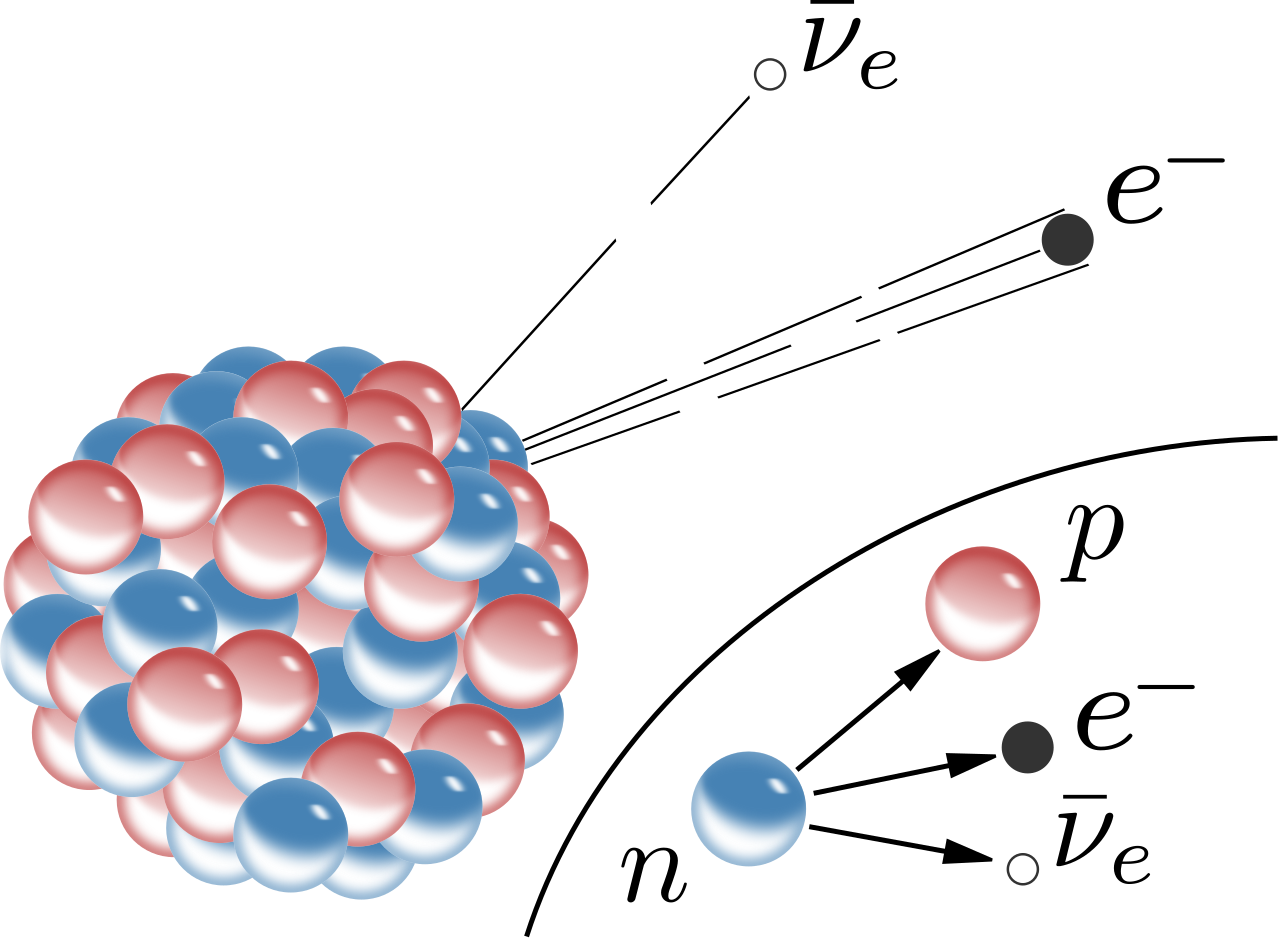
Antineutrinos are produced in the radioactive decay of the fission products. The fission products that are produced in the nuclear fission are often radioactive and therefore undergo a type of radioactive decay called β⁻ decay. In β⁻ decay, a neutron is transformed into a proton, thereby emitting an electron (also known as β-particle) and an antineutrino (see figure 2). Antineutrinos are the antiparticles of neutrinos, just as the positron is the antiparticle of the electron. Antineutrinos have almost the same properties as neutrinos. As mentioned earlier, neutrinos do not undergo much interaction with other particles, and so neither do antineutrinos. Therefore, the antineutrinos that are produced in the reactor easily escape the reactor core and travel over enormous distances. These (almost) freely traveling antineutrinos can in turn be detected by neutrino detectors, which is already shown by experiments such as the KamLAND detector in Japan, that is able to detect antineutrinos from nearby detectors.
Monitoring reactors
The antineutrinos from reactor processes can thus be detected hundreds of kilometers away from the reactor. But how can these neutrinos provide information on the activities within the reactor? This can be achieved in several ways.
The first method has to do with the energy spectra of the detected neutrinos. For nuclear energy production, while the reactor operates, the amount of U-235 decreases. In the meantime, other isotopes, which can also undergo fission, are created. This results in a broad range of antineutrinos, since each isotope undergoing fission produces a unique spectrum of antineutrinos. On the contrary, nuclear weapon production would, due to the high percentage of U-235, result in an antineutrino spectrum almost entirely dominated by U-235 fission, with only a small contribution from other isotopes. The detector can measure the energy spectra of the antineutrinos coming from a reactor. By analyzing the energy spectra of the detected antineutrinos, the proportions of isotopes that contribute to fission inside the reactor can be determined.
The second method involves the flux of the detected antineutrinos. The process of nuclear weapon production is mainly focused on maximizing the plutonium production, specifically Pu-239, while minimizing the production of Pu-240, another isotope of plutonium. Pu-240 has a high rate of spontaneous fission events and is therefore undesirable for nuclear weapons. To accomplish the production of the desired isotope only, the reactor operates for shorter cycles and is shut down frequently to extract the partially burned fuel. This results in fewer fission reactions overall and a lower and less constant neutrino flux compared to energy production reactors, where the reactor operates continuously for a long time. Measuring the flux of antineutrinos coming from a reactor can convince us that a reactor is not engaging in nuclear weapon production.
Challenges to implementation
Despite their potential, neutrino detectors face significant challenges as tools for reactor monitoring. For example, current neutrino detectors are very massive and expensive to build and maintain, making them impractical compared to simpler, established methods like on-site inspections. While current research in this area is promising, further enhancements are necessary to be able to implement this method in the future.
Another challenge is the interpretation and analysis of the data from neutrino detectors, which is very complex. Obtaining information on the activities within a reactor by analyzing the neutrino flux requires distinguishing the reactor neutrinos from background neutrinos coming from other sources. Moreover, antineutrinos emitted in the reactor come from several isotopes within the fuel, each having a specific spectral signature. When the detector measures these spectral data, it captures a superposition of the antineutrino spectra from all different isotopes in the fuel. Accurately identifying and attributing the measured spectra to specific isotopes or fuel compositions is crucial and also quite challenging.
The primary issue relates to the extremely low interaction rate of antineutrinos – at very large distances, the number of measured neutrinos in detectors can be as small as a single antineutrino per day. Therefore, highly sensitive and precise detectors, capable of discerning the low flux of antineutrinos emitted from reactors, are required. Due to this low interaction rate, the proximity of the detector to the reactor is another major limitation – most neutrino detectors must be placed within tens of meters of a reactor for effective monitoring. However, a recent discovery by the SNO+ detector in Sudbury, Canada provided a breakthrough in this problem: in 2023, this water-filled detector provided the first evidence of reactor antineutrinos in the detector, while the nearest reactor is located 240 km away from the detector. This suggests that large-scale water-based detectors could possibly be used for monitoring nuclear reactors from a large distance.
Overall, using neutrino detectors for reactor monitoring is currently not a realistic scenario, but definitely holds potential for the future. Researchers are actively working on developing smaller and less expensive neutrino detectors, which could make this technology more accessible. Advancements in detector material and data analysis techniques are expected to improve the sensitivity of neutrino detectors, thereby reducing background noise. In addition, collaborations of international institutions, such as the IAEA and research institutions, are very important to improve these techniques by sharing the available knowledge. If the current challenges can be overcome, neutrino detectors could become a powerful tool for verifying reactor activities, thereby improving global nuclear security and supporting non-proliferation efforts.
QU is sinds kort weer actief op Instagram! Volg ons voor nieuws en aankondigingen van nieuwe artikelen: https://www.instagram.com/quantumuniverse.nl/